Dennis W. Prather, Professor, Department of Electrical and Computer Engineering University of Delaware, USA
Published: 1 Jun 2016

CTN Issue: June 2016
A note from the editor:
This month we highlight new technology that uses optical holographic methods to literally image the signals coming off the massive MIMO antenna array, avoiding a lot of the difficult issues with ADC cost and beam processing. Could this be the missing technology to bring practical mMIMO to the field? We thought it was fascinating. Your comments are most welcome.
Alan Gatherer, Editor-in-Chief
Toward Holographic RF Systems for Wireless Communications and Networks
Dennis W. Prather, Professor, Department of Electrical and Computer Engineering University of Delaware, USA
This article introduces the notion of holographic RF systems for wireless communications as the ultimate limit in MIMO based networks. The idea is to build on the progression from single-user (SU) MIMO systems, where spatial diversity is introduced to render a more robust communication channel, to multi-user (MU) MIMO, where beam-space concepts are introduced to provide enhanced data capacity through parallel data transmission. In both cases pre-coding techniques can be used to account for channel-state-information (CSI) and render a more effective overall communication system. However, as the beam-space dimension approaches a continuum of plane waves with associated complex weights and CSI is also encoded as an additive set of complex weights, the complex wave amplitude across the transmitting aperture becomes a holographic profile, as the result of a collective superposition of the spatial-spectral frequencies within the communication environment as well as the anticipated interference resulting from scattering within the channel. To realize such a transmission aperture, namely, one capable of radiating holographic wave amplitude profiles, requires a dense array of transmitting elements that have continuous amplitude and phase control over the entire communication bandwidth, such that the far-field radiation pattern becomes identically matched to the overall requirements of the communication system.
To realize such a capability on the transmit, Tx, side requires a transmitting RF aperture that enables complete analog amplitude and phase control over a near continuum of radiating elements. Such a transmitting aperture is referred to as a current sheet [1] and can be realized using a tightly coupled array (TCA) of dipoles, or other elemental radiating elements [2]. In this article, we introduce such an array by using a coherent optical feed network that allows for a complete analog amplitude and phase control as well as high fidelity RF signal generation at each array element, such that the collective profile across the array represents the desired hologram for transmission.
On the receive, Rx, side this approach requires a processor that offers a continuum of spatially resolved and orthogonal signal-detection points. To this end, the receiver must be able to spatially resolve and process a continuum of received beams and locate them uniquely within an array of detector elements where each element corresponds to an independent angular sector from which the signals came. This operation is equivalent to an imaging process and can be realized using an up-converting phased array that relies on Fourier Optics concepts to perform spatial mapping of the RF environment to a corresponding detector array [3]. The details of both the Tx and Rx systems are presented below.
The notion of a current sheet as the ideal transmission aperture for RF signals has been a long sought goal, dating back to Harold Wheeler’s [1] and David Staiman’s [2] work in 1965 and 1968, respectively. While such apertures can be well approximated with tightly coupled arrays (TCAs) of dipoles, the dense feed network necessary to drive the antenna proved elusive. To address this challenge, we have developed an optically driven TCA that enables a close approximation to an ideal current sheet array antenna [4], see Figure 1. The idea here is to leverage progress in the development of high power, high linearity photodiodes [5-7] designed at the University of Virginia and fabricated at the University of Delaware. These detectors have been shown to deliver an output RF power of over 1 W at 10 GHz under continuous wave operation [8] and over 10 W peak power at 10 GHz using low duty cycle pulses [9].
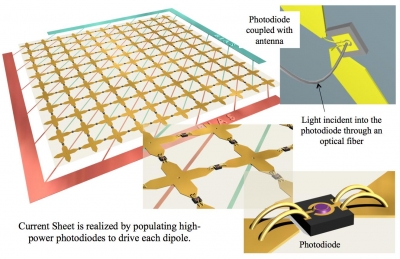
We use these detectors to realize a photodiode-coupled array antenna, which is an approximation to an ideal current sheet array (CSA), where the current sources exciting the coupled dipole elements are photodiodes. This antenna design provides maximized capabilities for relative bandwidth, scanning angle, and low profile, due to the inherent planar dipole structure, and potentially unlimited bandwidth of the CSA. Additionally, the fundamental nature of the TCA approximation to the CSA is the large element oversampling, which, combined with the small photodiode chip size (0.5mm x 0.5mm), sets the photodiode bandwidth as the upper operational frequency.
To drive the TCA, we have developed an optical-feed based on two phase-locked lasers that are "off-set" in frequency. The difference frequency is the RF carrier to be transmitted by the array and because the two lasers are phase-locked the optical phase noise cancels, which results in high fidelity RF signal generation down to 1 Hz line-width [10]. In this setup, the phase-locked lasers are split into N fibers and each fiber is routed to a unique photodetector, which is directly connected to the feed points of a given antenna element in the TCA, compare Fig. 1. Shifting the phase of the output of one of the laser relative to the other imposes a commensurate phase shift on the RF signal generated by the optical downconversion, thereby achieving fully analog RF beam forming across the TCA. In addition, this approach can realize very complex waveforms that contain extremely high data rates (we have demonstrated up to 256 QAM with this approach) and fully account for the CSI. Moreover, this can all be done on multiple RF carriers in parallel, with simultaneous beam-forming, and incorporating CSI, thereby realizing a true holographic transmission profile that accounts for the unique aspects of the radiation characteristics of the communication environment.
On the Rx side, we have developed an RF receiver that first spatially processes all of the received signals and then processes them temporally, see Figure 2. Accordingly, the signals are first spatially separated onto different detectors and then individually processed as received signals, which serves to mitigate signal intermixing and intermodulation thereby allowing for extensive frequency re-use across spatial sectors.
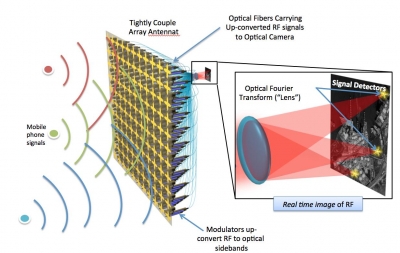
To help understand this concept, imagine a “big” lens that exists in front of a base station that would literally image all of the received signals to an array of RF detectors. Now consider that there are many antennas/detectors within the array (like a focal plane array in a camera) and each one receives signals that correspond to a particular spatial direction, or angle-of-arrival, as it is focused by the lens. Such approaches have actually been implemented with Luneburg lenses, and have shown significant increases in data capacity [11]. In this process of “imaging” the RF signal environment, spatial orthogonality is achieved, which mitigates ACI and CCI by preventing the interaction of signals originating at different spatial locations.
To realize this capability, we developed a phased array receiver system, where each element in the array is connected to an optical mixer, or modulator, that converts the received RF signal into the sideband of an optical carrier. After this collective up-conversion, the optical signals are gathered into a common fiber bundle (where the location of each fiber matches the location of the antenna element to which it is connected in the array), the sidebands are filtered off of the carrier and launched into free-space to allow for all of the optical beams to overlap where they are subsequently “imaged” with an optical lens onto a photo-detector array with each photo-detector corresponding to a unique spatial direction. The original optical carrier is then used to mix the focused optical sidebands on each photo-detector to regenerate the baseband RF signal for network processing. Using this approach, we have demonstrated several hundred Mbps and up to 256 QAM.
The unique aspect of our approach is that we use optical "up-conversion" to allow for the use of an optical Fourier-transform lens to spatially process the complex RF signals over the entire array-antenna aperture. In so doing, we achieve a spatial processor for a near continuum of RF beams that literally performs an analog Inverse Fourier transform at the speed of light with a potentially unlimited beam-bandwidth product. Perhaps the main advantage of this approach is that it allows for the spatial processing of all the RF waves in the communication environment prior to actual signal detection, which alleviates any interaction between signals and thus inhibits signal intermixing and interaction. Ultimately, this enables frequency re-use on a massive scale as each sector can use the entire spectral band while the spatial orthogonality between the sectors ensures that they operate with little to no CCI or ACI. We call this system an "imaging" receiver because it literally "images" the RF scene by spatially mapping every received RF signal onto a particular detector based on its point of origin in the environment.
Another unique feature of the imaging receiver approach, in comparison to those that use RF lenses, e.g., Luneburg, is that it provides the means to encode complex phase profiles across the Rx array aperture thereby enabling the use of a “matched” spatial filter to mitigate multi-path effects and, consequently, account for full CSI in each received signal. Moreover, by using an optical Fourier transform lens, we can perform all of the spatial processing on the up-converted RF signals simultaneously without the use of an analog-to-digital converter. The end result is the ability to spatially process the received RF signals in an optimal way that maximizes the use of communication system.
In summary, we have introduced a new underlying hardware architecture that allows for a fully analog Tx holographic transmission and Rx spatial processing capability for wireless communication systems. This enables a near continuum of spatial-spectral precoding and processing with high throughput. In the limit, as the this domain becomes a continuous waveform, the Tx and Rx antenna apertures become holographic profiles and thereby require RF apertures capable of encoding them to achieve optimal link performance.
References
- H. A. Wheeler, “Simple Relations Derived from a Phased-Array Antenna Made of an Infinite Current Sheet,” IEEE Transactions on Antennas and Propagation, pp. 506-514, July 1965.
- D. Staiman, M. E. Breese, and W. T. Patton, “New Technique for Combining Solid-State Sources,” IEEE Journal of Solid-State Circuits, vol. SC-3, No. 3, September 1968.
- R. D. Martin; S. Shi; Y. Zhang; A. Wright; P. Yao; K. P. Shreve; C. A. Schuetz; T. E. Dillon; D. G. Mackrides; C. E. Harrity, and D. W. Prather, “Video rate passive millimeter-wave imager utilizing optical upconversion with improved size, weight, and power,” Proc. SPIE 9462, Passive and Active Millimeter-Wave Imaging XVIII, 946209, May 2015.
- J. Bai, S. Shi, G. J. Schneider, J. P. Wilson, Y. Zhang, W. Pan, and D. W. Prather, “Optically Driven Ultrawideband Phased Array With an Optical Interleaving Feed Network,” IEEE Antennas and Wireless Propagation Letters, vol. 13, pp. 47–50, 2014.
- X. Xie, Q. Zhou, K. Li, A. Beling, and J. C. Campbell, “High performance analog photonic link based on modified uni-traveling-carrier photodiode,” in 2014 Conference on Lasers and Electro-Optics (CLEO),2014, pp. 1–2.
- Z. Yang, X. Xie, Q. Li, J. C. Campbell, and A. Beling, “20 GHz analog photonic link with 16 dB gain based on a high-power balanced photodiode,” in Photonics Conference (IPC), 2015, 2015, pp. 144–145
- Z. Li, Y. Fu, M. Piels, H. Pan, A. Beling, J. E. Bowers, and J. C. Campbell, “High-power high-linearity flip-chip bonded modified uni-traveling carrier photodiode,” Opt. Express, vol. 19, no. 26, pp. B385–B390, 2011.
- X. Xie, Q. Zhou, K. Li, Y. Shen, Q. Li, Z. Yang, A. Beling, and J. C. Campbell, “Improved power conversion efficiency in high-performance photodiodes by flip-chip bonding on diamond,” Optica, vol. 1, no. 6, p. 429, Dec. 2014.
- X. Xie, K. Li, Y. Shen, Q. Li, J. Zang, A. Beling, and J. C. Campbell, “Photonic Generation of High-Power Pulsed Microwave Signals,” J. Lightwave Technol., JLT, vol. 33, no. 18, pp. 3808–3814, Sep. 2015.
- G. J. Schneider, J. A. Murakowski, C. A. Schuetz, S. Shi, and D. W. Prather, “Radiofrequency signal-generation system with over seven octaves of continuous tuning,” Nat Photon, vol. 7, no. 2, pp. 118–122, Feb. 2013.
- http://www.wirelessweek.com/news/2015/03/t-deploying-giant-ball-boost-capacity-sxsw
Biography
Dennis Prather began his professional career by joining the US Navy in 1982, where he still serves in the reserves and serves as a CAPT (O-6) Engineering Duty Officer. After active duty, he received the BSEE, MSEE, and PhD from the University of Maryland in 1989, 1993, and 1997, respectively. During this time he worked as a senior research engineer for the Army Research Laboratory, where he performed research on both optical devices and architectures for information processing. His efforts included work on the modeling, design, and fabrication of meso-scale optical elements and their integration with active opto-electronic devices, such as semiconductor lasers and focal plane arrays. In 1997 he joined the Department of Electrical and Computer Engineering at the University of Delaware. Currently he is the College of Engineering Distinguished Professor and his research focuses on both the theoretical and experimental aspects of RF-photonic elements and their integration into various systems for imaging, communications and Radar. To achieve this, his lab develops computational electromagnetic models and fabrication/integration processes necessary for the demonstration of state-of-the-art RF-photonic devices such as: ultra-high bandwidth modulators, silicon photonic RF sources, photonic crystal chip-scale routers, meta-material antennas, and integrated RF-Photonic phased arrays.
Professor Prather is currently an Endowed Professor of Electrical Engineering, he is a senior member of the IEEE, Fellow of the Society of Photo-Instrumentation Engineers (SPIE) and a Fellow of the Optical Society of America (OSA). He has authored or co-authored over 500 scientific papers, holds over 20 patents, and has written 14 books/book-chapters.
Statements and opinions given in a work published by the IEEE or the IEEE Communications Society are the expressions of the author(s). Responsibility for the content of published articles rests upon the authors(s), not IEEE nor the IEEE Communications Society.