Timothy Creazzo and Dennis W. Prather
Published: 25 Oct 2023

CTN Issue: October 2023
A note from the editor:
As the fall season descends upon us, our October article takes us on a hayride through the somewhat unfamiliar yet fascinating landscape of photonic integrated circuits (PIC). Our October 2021 article, “Can RF Photonics Save the Wireless Day? Unbounded Beam-Bandwidth Product to the Rescue”, introduced the ComSoc community to the research and development efforts on RF photonic phased arrays, where photons interact with electrons to support extremely high beam-bandwidth applications for sensing and communication, envisaged by, e.g., the beyond-5G roadmap. Two years in, the authors are keen to share progress made in reducing the size, weight, power consumption and cost (SWAP-C) of such systems by way of PIC, bringing the approach closer to reality. This article provides an overview of the burgeoning foundry ecosystem for photonic integration, with a focus on the material platform frontrunners and challenges in heterogenous integration of electronic and photonic components. As always, reader comments and feedback are greatly appreciated.
Xiao Feng Qi, CTN Associate Editor
Photonic Integrated Circuits: Silicon-Adjacent Devices for High Beam-Bandwidth Communication and Sensing
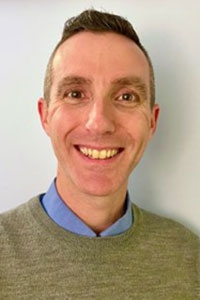
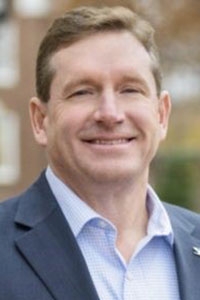
Dennis W. Prather
Professor of Electrical and Computer Engineering, University of Delaware and President, Phase Sensitive Innovations
Introduction
With the commercial implementation of 5G networks in full swing, service providers are striving for higher speed and lower latency data connections, to address the perpetually growing customer demand. Accordingly, higher radio frequencies (RF) and wider bandwidth massive MIMO systems are being developed for operational frequencies extending well into the millimeter wave (mmW) region of the spectrum and beyond for integrated sensing and communication (ISAC). Furthermore, 3GPP roadmaps have identified major enhancements in the areas of artificial intelligence (AI) and extended reality (XR) that are expected to be implemented in the 2025 time frame [1].
The move to higher frequencies and wider bandwidths is putting significant stress on the corresponding RF components required to implement such advanced systems. To this end, recent developments in the field of radio-frequency-photonics (RF-photonics) may offer a viable alternative to supporting the desired implementations not only in the near-term, but long into the future. This arises with the ultra-wide carrier frequency range of RF-photonic devices and systems and their inherently large bandwidths that serve to enable previously unattainable performance using solely RF technologies. As an example, an RF phased array beamformer employing intermediatory optical processing is proposed in [2]–[4], and illustrated in Figure 1. Optical up-conversion directly at each element in the RF front-end by means of electrooptical modulator (EOM) produces optical outputs that are gathered into a fiber bundle that forms an array to re-launch the up-converted RF signals back into free space, where they re-form the exact beams that were incident on the front-end antenna array. Re-forming of the RF beams in the optical domain is possible due to the spatially coherent up-conversion process, which preserves not only the amplitude and phase of the RF signal at each antenna element, but also the phase relations between the elements. At this point, an optical lens performs a real-time, no-latency, no-power, two-dimensional (2D) spatial Fourier transformation, which focuses each incident wavefront to a unique spot, see Figure 1. Each spot is then coupled through a lenslet to a corresponding fiber that routes the optical signal to a high-speed photodetector (PD, not shown), where it is mixed with an optical local-oscillator for down-conversion to an intermediate frequency (IF) of choice for further digital processing. Due to the extremely wide bandwidth of the EOM and PD, the RF photonic approach offers unprecedented performance in terms of beam-bandwidth product with significantly lower power consumption. Successful applications in sensing (e.g., passive mmWave imaging [3]. PIC based imaging sensor and atmospheric RF spectrometer are also in the works) and wireless communication (e.g., multi-user massive MIMO at mmWave range [4]) have been demonstrated. It is worth noting that while Fourier lens is suitable for far-field beamforming, it is conceivable that the optical upconversion approach can be applied to near-field wavefront manipulation as well, with the lens replaced by more versatile optical processing based on holographic principles.
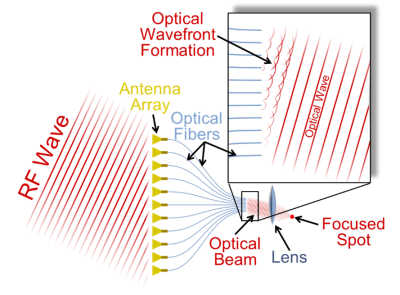
This article explores how photonic integrated circuits (PICs) can further improve the appeal of these optical systems as they offer significant reductions in size and weight. Herein we focus on the suitability of different material platforms for the functions required in the RF-photonic system and provide an example demonstration of a PIC capable of massively parallel beamforming at low power consumption.
Photonic Integrated Circuits (PICs) for RF-Photonic Imaging Systems
PICs are chips that contain multiple photonic devices and are the optical equivalent to electronic or RF integrated circuits (RFIC). The success of PICs has relied on the successful development of fundamental building blocks including lasers, modulators, waveguides, and photodiodes that allow for an optical source, electrical-to-optical conversion, signal routing, and optical-to-electrical conversion, respectively. For a wide array of applications, PICs can be realized by combining all or some of the respective fundamental building blocks. In addition, they can be implemented in a variety of material platforms with two of the most popular being Indium Phosphide (InP) and Silicon. InP is one of a few materials that can offer true monolithic integration of all the fundamental building blocks owing to its direct bandgap and common use for lasers and photodiodes. Silicon photonics emerged as a competitive platform due to its promise to leverage existing CMOS manufacturing infrastructure and landscape to allow for scaling to higher volumes and reach lower costs. This led to the research, development, and demonstration of reliable implementations of lasers and photodiodes using heterogeneous approaches in silicon that are compatible with CMOS foundry manufacturing [5]–[8]. Research on the development and improvement of fundamental building blocks across all material platforms continues today. Additionally, the emergence of multi-project wafer (MPW) shuttle runs for PICs over the last 10 to 15 years have provided lower cost and better accessibility to PIC development for researchers and industry alike. Naturally, the development of foundry specific process design kits (PDKs) has accompanied the MPW offerings leading to an increase in fabless PIC manufacturing business models and accelerating the development of more complex PICs across a wide range of applications.
Although one of the main drivers for PIC development is optical interconnects, with a focus on data communications [9], the availability of reliable PDKs with a wide range of devices well beyond the fundamental building blocks provides an opportunity for expanding the application of PICs to other areas of the network architecture that notably includes RF processing at or near the wireless antenna. In a past article, an RF-Photonic phased array system was presented that offers a unique capability to achieve a near unlimited beam-bandwidth product enabled by photonic devices [2]. These RF-Photonic phased array systems implement wavefront reconstruction using optics, and a more complete description of how those systems work can be found in [3], [4].
It is easy to see how an invaluable reduction in size and weight could result from the conversion of the bulk or discrete optical components to PICs for the RF photonic phased array system. The goal for replacing all optical functionality in a system such as this involves hybrid or heterogeneous integration to leverage the best material for each function in the system; however, a closer inspection reveals a natural development path to leverage more mature foundry capabilities. More specifically, optical components performing the upconversion in an RF-photonic system (e.g., electro-optic modulator (EOM)) typically sit close to the antenna, but the remaining optical processing elements can be remoted via optical fiber. This natural segregation of optical elements that also corresponds to functionality lends itself extremely well to PIC development from a materials perspective. For the upconversion modulators, lithium niobate EOMs provide extremely high performance supporting upconversion of RF signals well beyond 100 GHz [10]. The separation of this functionality from the additional optical processing PIC, paired with a direct down conversion approach to IF, alleviates the near-term need for high RF bandwidths in the optical processing PIC. This allows the optical processing PIC to be realized monolithically in another single material platform such as silicon. The separation of functionality by material enables the leveraging of mature PIC platforms that do not require significant process development (i.e., silicon photonics), which leads to an ability to focus on understanding the performance and implementation requirements of complex PICs that enable tangible benefits in deployed RF processing systems. The next section provides a more detailed evaluation of how four major PIC material platforms compare for the development of RF-Photonic system implementation.
RF-Photonic PICs: Material Platform Summary
As mentioned above, the material selection for the PIC elements in an RF-photonic system is aided by the natural separation between functional blocks of the system. With a goal of selecting the best material for each major functional element of the presented RF-photonic system, we consider the capability of each material as well as the maturity and availability for realization of a reliable PIC. It is important to note, as mentioned previously, hybrid and heterogeneous integration approaches may comprise alternative platforms to attain the highest levels of integration in systems that rely on more than one material for optimal performance. While heterogeneous and hybrid solutions are not excluded from our long-term roadmaps, the subsequent comparisons are intended to identify robust near-term solutions for PIC implementations that provide significant impacts on the system size, weight, power, and cost (SWaP-C).
Four core material platforms are evaluated as potential materials for the development of the PICs: Silicon/Silicon Nitride, III-V compounds (i.e., InP), Lithium Niobate (LN), and thin-film LN. Table 1 includes a summary of the performance and capability for each of these platforms across a range of categories that pertain to PIC development. Each category represents a significant building block for photonic integration (i.e., lasers, modulators, detectors, and fiber coupling) or a critical capability when considering PIC development both generally and for RF applications (i.e., bandwidth, size/complexity, accessibility, and power). The power category refers to optical power handling capability as opposed to power consumption. The chosen set of categories is not intended to be all inclusive. Subsequent sections elaborate on each of the candidate materials with reference to the categories in the table.
Material Platform | Bandwidth | Size/ Complexity |
Accessibility | Lasers | Modulators | Detectors | Fiber Coupling | Power |
---|---|---|---|---|---|---|---|---|
Silicon | B | A | A | C | B | A | A | B |
InP | A | B | C | A | B | A | A | B |
Thin Film LN | A | A | B | C | A | C | B | A |
Bulk LN | A | C | B | C | A | C | A | A |
Silicon/Silicon Nitride (Si/SiN)
The primary silicon photonics platform consists of silicon-on-insulator (SOI) that leverages the large optical index contrast between silicon and silicon dioxide to provide highly confined optical waveguides. Silicon photonics leverages the existing CMOS foundry infrastructure to provide PICs with very high levels of complexity, and in some cases, integration with CMOS electronics has been demonstrated [11]. Accordingly, access to reliable foundries with PDKs for silicon photonics has become more common, and some current choices include: AIM Photonics, IMEC, IME, GlobalFoundries, INPHOTEC, TowerJazz, LioniX, Sandia National Labs, and LIGENTEC [12], [13], to name a few. Silicon nitride (SiN) has been included by extension since Si and SiN are compatible with one another and both can be processed using CMOS technologies. For the listed entities offering a PDK and MPW runs, some offer silicon only, others silicon nitride only, yet others both. The AIM Photonics Manufacturing Institute is one of the newest foundry that has both silicon and silicon nitride with a relatively large PDK offering [14].
The combination of readily accessible development options supporting high levels of complexity but limited bandwidth uniquely positions silicon PICs for handling RF-photonic system functionalities such as the optical processing in a RF-photonic phased array system. The optical processing functionality benefits significantly from high complexity, which supports future scaling to systems with high channel counts, while it does not require high frequency operation (the most demanding bandwidth requirement is the photodetectors, which only need to support direct down conversion to IF).
Indium Phosphide (InP)
The most popular III-V material platform of interest for a majority of PIC applications including RF-photonics is Indium Phosphide (InP), which supports light generation capabilities near 1.55 µm. Clearly, the main advantage of the InP platform is the ability to monolithically integrate lasers at the chip-scale [15]. Owing to this ability, an industry and corresponding infrastructure has been developed for wafer-scale processing of InP that includes all the necessary fundamental photonic device building blocks [16], [17]. Much of the developed high performance device building blocks (i.e., lasers, modulators, and photodetectors) is attributable to the optoelectronic industry; however, many of the prescribed devices have been developed individually. The photonic InP platform is based on epitaxial growth of materials specific to the desired device (i.e. a different type of epitaxial growth each for lasers, modulators and detectors), which makes high levels of integration and complexity extremely difficult and costly to achieve [18]. As such, the availability of InP-based photonic PDKs and MPW runs is considerably scarcer in comparison to silicon. The joint European platform for photonic integrated components and circuits (JePPIX) offers photonic InP MPW services, and more recently, Sandia National Labs has an offering that may be accessible through AIM Photonics [19].
The lack of access to MPW options for development of PICs in InP is the main reason for the red color code under the accessibility category in Table 1. Beyond the accessibility hurdle, the performance and device capabilities do provide an option for development of PICs in InP for RF-photonic applications.
Lithium Niobate and Thin Film Lithium Niobate
Lithium Niobate has long been a preferred material for analog optical RF applications, and specifically for EOMs, due to its superior electro-optic performance [20]. This performance has led to the development and commercial availability of optical modulators with bandwidth performance that is unmatched by other material systems and, furthermore, an industry infrastructure for producing such devices. Although several companies maintain lithium niobate specific foundries for their own product development, no MPW services currently exist. The lack of available MPW services presents a major obstacle to PIC development in lithium niobate.
The optical upconversion modulator within the RF-photonic phased array system benefits from development as an individual device due to the architecture breakdown of the system (as mentioned above). More specifically, positioning the modulator as closely as possible to the antenna while also allowing it to be separate from the remainder of the optical processing hardware maximizes the benefits of switching from RF processing to optical processing by minimizing the amount of RF components required and supporting flexibility in the configuration of the RF front-end (again, especially at lower frequencies where phased array antennas may be large).
The optical upconversion performance benefits of lithium niobate modulators have fueled the demonstration of RF photonic phased arrays systems, like the one described above, as well as passive mmW imagers, multi-channel transmit and receive systems, and RF photonic links. Nonetheless, the continued demand for better performance and higher levels of integration has led to a transition into the thin-film lithium niobate device development to pursue better performance and higher levels of integration.
The limitations on dense integration of bulk lithium niobate are due mainly to the low index contrast waveguides. Thin-film lithium niobate overcomes this limitation by combining lithium niobate with an oxide (typically silicon dioxide) to enable the development of higher index contrast waveguides. Higher index contrast waveguides in lithium niobate not only support higher levels of complexity in integration but also provide significant performance improvements to modulator devices. The reduction in the size of the optical mode allows for better overlap with the applied electric field for modulation, which leads to an improvement in the device efficiency (effectively a reduction of the half-wave voltage for modulation), and the increased presence of oxide improves the index matching between the RF and optical signals leading to improved modulation bandwidth [10], [21].
Although accessibility to fabrication capabilities is typically limited for lithium niobate, recent research and development, including heterogeneous integration of lasers, has shown that thin-film lithium niobate may be a considerably competitive platform for future RF-photonic systems [20], [22], [23].
An RF-Photonic Beamforming PIC
Based on the evaluation of system functionality and compatibility with available mature PIC development platforms, the demonstration of an optical processing PIC for a RF phased array system has been made possible using silicon photonics, as presented in Figure 2 [24], [25]. Figure 2(a) shows a schematic of an RF imaging system with optical processing that is receiving two different RF waves. The optical processing of the two RF waves produces spots at two different locations at the output dependent on their angles of arrival. Figure 2(b) and (c) show measured infrared images of the output of the optical processing PIC corresponding to two different RF waves as shown in Figure 2(a) noting that each incoming RF wave maps to a different output waveguide of the PIC. The optical processing PIC enabling this demonstration is shown in Figure 2(d), which only occupies a footprint of 0.25 by 0.15 inches. Figure 2(e) shows the same PIC after packaging with optical fibers and connections to electronic controls in a 2.1- by 1.6-inch aluminum housing.
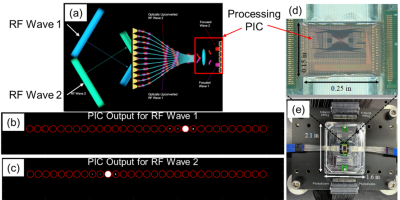
Conclusion
RF-photonic techniques implemented through PICs may be well positioned to provide future-proof architectural solutions for high beam-bandwidth networks envisioned by beyond-5G network evolution. Access to PIC development through mature MPWs and/or dedicated foundry processes with corresponding PDKs provides an opportunity for RF-minded designers to explore the possibilities in every aspect of the network architecture. Although PDKs and foundry process for PICs have matured in the last decade, challenges still exist with regards to obtaining maximum benefits from the implementation of PICs in RF systems and elsewhere. Major challenges revolve around packaging and integration of PICs with supporting electronic and RF integrated circuits. The accelerating research and development efforts on heterogeneous integration will enable highly integrated RF photonic modules that mix and match the best performing material system for each of the required system functions, paving the way for PICs to become an integral part of RF communication and sensing systems.
References
- “Becoming 5G Advanced: The 3GPP 2025 Roadmap.” Dec. 2022. [Online]. Available: https://www.5gamericas.org/wp-content/uploads/2022/12/Becoming-5G-Advanced-the-3GPP-2025-Roadmap-InDesign.pdf
- “Can RF Photonics Save the Wireless Day? Unbounded Beam-Bandwidth Product to the Rescue. | IEEE Communications Society.” Accessed: Sep. 13, 2023. [Online]. Available: https://www.comsoc.org/publications/ctn/can-rf-photonics-save-wireless-day-unbounded-beam-bandwidth-product-rescue
- D. W. Prather et al., “Millimeter-Wave and Sub-THz Phased-Array Imaging Systems Based on Electro-Optic Up-Conversion and Optical Beamforming,” IEEE J. Sel. Top. Quantum Electron., vol. 29, no. 5: Terahertz Photonics, pp. 1–14, Sep. 2023, doi: 10.1109/JSTQE.2023.3306953.
- D. W. Prather et al., “Fourier-Optics Based Opto-Electronic Architectures for Simultaneous Multi-Band, Multi-Beam, and Wideband Transmit and Receive Phased Arrays,” IEEE Access, vol. 11, pp. 18082–18106, 2023, doi: 10.1109/ACCESS.2023.3244063.
- Z. Zhou et al., “Prospects and applications of on-chip lasers,” eLight, vol. 3, no. 1, p. 1, Jan. 2023, doi: 10.1186/s43593-022-00027-x.
- A. W. Fang, H. Park, O. Cohen, R. Jones, M. J. Paniccia, and J. E. Bowers, “Electrically pumped hybrid AlGaInAs-silicon evanescent laser,” Opt. Express, vol. 14, no. 20, Art. no. 20, 2006.
- T. Creazzo et al., “Integrated tunable CMOS laser,” Opt. Express, vol. 21, no. 23, Art. no. 23, 2013.
- C. T. DeRose et al., “Ultra compact 45 GHz CMOS compatible Germanium waveguide photodiode with low dark current,” Opt. Express, vol. 19, no. 25, p. 24897, Dec. 2011, doi: 10.1364/OE.19.024897.
- P. I. C. Magazine, “Datacom will be the main driver for Silicon Photonics - PIC Magazine News,” PIC (Photonics Integrated Circuits). Accessed: Sep. 13, 2023. [Online]. Available: https://picmagazine.net/article-gen/117150
- A. J. Mercante, S. Shi, P. Yao, L. Xie, R. M. Weikle, and D. W. Prather, “Thin film lithium niobate electro-optic modulator with terahertz operating bandwidth,” Opt. Express, vol. 26, no. 11, p. 14810, May 2018, doi: 10.1364/OE.26.014810.
- A. H. Atabaki et al., “Integrating photonics with silicon nanoelectronics for the next generation of systems on a chip,” Nature, vol. 556, no. 7701, pp. 349–354, Apr. 2018, doi: 10.1038/s41586-018-0028-z.
- S. Y. Siew et al., “Review of Silicon Photonics Technology and Platform Development,” J. Light. Technol., vol. 39, no. 13, pp. 4374–4389, Jul. 2021, doi: 10.1109/JLT.2021.3066203.
- A. E.-J. Lim et al., “Review of Silicon Photonics Foundry Efforts,” IEEE J. Sel. Top. Quantum Electron., vol. 20, no. 4, pp. 405–416, Jul. 2014, doi: 10.1109/JSTQE.2013.2293274.
- N. M. Fahrenkopf, C. McDonough, G. L. Leake, Z. Su, E. Timurdogan, and D. D. Coolbaugh, “The AIM Photonics MPW: A Highly Accessible Cutting Edge Technology for Rapid Prototyping of Photonic Integrated Circuits,” IEEE J. Sel. Top. Quantum Electron., vol. 25, no. 5, pp. 1–6, Sep. 2019, doi: 10.1109/JSTQE.2019.2935698.
- J. Klamkin et al., “Indium phosphide photonic integrated circuits: Technology and applications,” in 2018 IEEE BiCMOS and Compound Semiconductor Integrated Circuits and Technology Symposium (BCICTS), IEEE, 2018, pp. 8–13.
- G. E. Hoefler et al., “Foundry Development of System-On-Chip InP-Based Photonic Integrated Circuits,” IEEE J. Sel. Top. Quantum Electron., vol. 25, no. 5, pp. 1–17, Sep. 2019, doi: 10.1109/JSTQE.2019.2906270.
- R. Nagarajan et al., “InP Photonic Integrated Circuits,” IEEE J. Sel. Top. Quantum Electron., vol. 16, no. 5, pp. 1113–1125, Sep. 2010, doi: 10.1109/JSTQE.2009.2037828.
- L. A. Coldren et al., “High Performance InP-Based Photonic ICs—A Tutorial,” J. Light. Technol., vol. 29, no. 4, Art. no. 4, Feb. 2011, doi: 10.1109/JLT.2010.2100807.
- “InP Multi-Project Wafers,” AIM Photonics. Accessed: Sep. 13, 2023. [Online]. Available: https://www.aimphotonics.com/inp-mpw
- J. E. Toney, Lithium niobate photonics. in Artech House applied photonics series. Boston: Artech House, 2015.
- A. N. R. Ahmed, S. Shi, M. Zablocki, P. Yao, and D. W. Prather, “Tunable hybrid silicon nitride and thin-film lithium niobate electro-optic microresonator,” Opt. Lett., vol. 44, no. 3, p. 618, Feb. 2019, doi: 10.1364/OL.44.000618.
- X. Liu, X. Yan, Y. Liu, H. Li, Y. Chen, and X. Chen, “Tunable single-mode laser on thin film lithium niobate,” Opt. Lett., vol. 46, no. 21, p. 5505, Nov. 2021, doi: 10.1364/OL.441167.
- L. Chang et al., “Heterogeneous integration of lithium niobate and silicon nitride waveguides for wafer-scale photonic integrated circuits on silicon,” Opt. Lett., vol. 42, no. 4, p. 803, Feb. 2017, doi: 10.1364/OL.42.000803.
- D. Prather et al., “Optically Upconverted, Spatially Coherent Phased-Array- Antenna Feed Networks for Beam-Space MIMO in 5G Cellular Communications,” 2023.
- D. W. Prather and C. A. Schuetz, “Photonic Integrated Circuits in RF Beamforming for Optimized 5G/6G Wireless Communications,” in 2023 IEEE Research and Applications of Photonics in Defense Conference (RAPID), Miramar Beach, FL, USA: IEEE, Sep. 2023, pp. 1–2. doi: 10.1109/RAPID54473.2023.10264721.
Statements and opinions given in a work published by the IEEE or the IEEE Communications Society are the expressions of the author(s). Responsibility for the content of published articles rests upon the authors(s), not IEEE nor the IEEE Communications Society.